Growth Factor Content in Human Sera Affects the Isolation of Mesangiogenic Progenitor Cells (MPCs) from Human Bone Marrow
- 1Department of Clinical and Experimental Medicine, University of Pisa, Pisa, Italy
- 2Department of Surgical, Medical and Molecular Pathology and Critical Care Medicine, University of Pisa, Pisa, Italy
- 3Department of Translational Research and New Technologies in Medicine and Surgery, University of Pisa, Pisa, Italy
Mesangiogenic Progenitor Cells (MPCs) are human bone marrow-derived multipotent cells, isolated in vitro under selective culture conditions and shown to retain both mesengenic and angiogenic potential. MPCs also co-isolated with multipotent stromal cells (MSCs) when bone marrow primary cultures were set up for clinical applications, using human serum (HS) in place of fetal bovine serum (FBS). MPC culture purity (over 95%) is strictly dependent on HS supplementation with significant batch-to-batch variability. In the present paper we screened different sources of commercially available pooled human AB type serum (PhABS) for their ability to promote MPC production under selective culture conditions. As the majority of “contaminating” cells in MPC cultures were represented by MSC-like cells, we hypothesized a role by differentiating agents present in the sera. Therefore, we tested a number of growth factors (hGF) and found that higher concentrations of FGF-2, EGF, PDGF-AB, and VEGF-A as well as lower concentration of IGF-1 give sub-optimal MPC recovery. Gene expression analysis of hGF receptors was also carried out both in MSCs and MPCs, suggesting that FGF-2, EGF, and PDGF-AB could act promoting MSC proliferation, while VEGF-A contribute to MSC-like cell contamination, triggering MPC differentiation. Here we demonstrated that managing hGF contents, together with applying specific receptors inhibitors (Erlotinib-HCl and Nintedanib), could significantly mitigate the batch-to-batch variability related to serum supplementation. These data represent a fundamental milestone in view of manufacturing MPC-based medicinal products.
Introduction
Mesenchymal stromal cells (MSCs) are multipotent adult progenitor cells isolated in vitro from a plethora of different tissues (reviewed in Murray et al., 2014) as their perivascular origin has been demonstrated and widely accepted (da Silva Meirelles et al., 2006; Crisan et al., 2008). MSC role as skeletal tissue progenitors has been investigated in a variety of studies in animal models, and cell-based therapy approaches demonstrated their beneficial effects in the regeneration of damaged skeletal tissues including bone (Watson et al., 2014), cartilage (Richardson et al., 2015), tendons (Pacini et al., 2007), and meniscus (Yu et al., 2015). For those reasons, most of the tissue engineering strategies and regenerative medicine approaches, developed to de novo skeletal tissue formation, involved MSCs (Yousefi et al., 2016). Nonetheless, it has been reported that an effective tissue remodeling response is in tight correlation with the formation of vascularized tissue (Rao and Stegemann, 2013; Hutton and Grayson, 2014). Commonly, the vascularization of the newly formed tissues in vivo has been expected driven in large part by the host response to the implant, and trigged by hypoxic condition surrounding the grafted cells (Chamberlain et al., 2015). Nonetheless, this effect could be hypothesized in acute injuries with moderate tissue loss, but in case of large implants or poor blood supply, as in non-union fracture or pseudoarthrosis (Hak et al., 2014), the vascularization of constructs would be compromised. Modular constructs obtained applying both osteoprogenitor cells, as MSCs, and endothelial cells (ECs) or their precursors (EPCs), have been investigated as an option in order to sustain vascularization of the implants, independently from the host response (Butler and Sefton, 2012). However, MSCs have been estimated to represent around 0.001–0.01% of human bone marrow mononuclear cells (hBM-MNCs) (Subbanna, 2007; Lechanteur et al., 2016), and similarly ECs and EPCs represent rare populations in their tissues of origin (Pelosi et al., 2014), thus pre-clinical and clinical applications of these cells, alone or in combination, require extensive ex vivo cell expansion to obtain therapeutic cell doses. Most widely accepted MSC isolation and expansion protocols, from bone marrow, are based on basal media supplemented with fetal bovine serum (FBS) (Haynesworth et al., 1992; Prockop, 1997). Similarly, consistent fractions of FBS should be applied also in the EC and EPC isolation (Kirton and Xu, 2010). However, all supplements of animal origin expose patients to a number of risks and represent a major obstacle to comply with good manufacturing practice (GMP) guidelines (Herberts et al., 2011). The urgent need for FBS alternatives led to consider human serum (HS) as the straightforward substitute in clinical-grade MSC production (Altaie et al., 2016).
In 2009, during the attempt to produce multipotent mesenchymal stromal cells (MSCs) in xeno-free GMP-compliant culture conditions, we had shown the occurrence of mesodermal (currently renamed “mesangiogenic”) progenitor cells (MPCs), in hBM-MNC cultures when using autologous serum (AS) (Petrini et al., 2009) or pooled human AB type serum (PhABS) (Trombi et al., 2009). MPCs have been described in vitro as slow cycling MSC progenitors that also retain angiogenic properties (Fazzi et al., 2011). They are characterized by peculiar fried egg-shape morphology, expression of pluripotency-associated markers OCT4 and NANOG, and intense nestin expression (Pacini et al., 2010). MPCs lack mesenchymal-associated markers CD73, CD90, and CD105 while showing occurrence of CD31, CD11c, and CD18.
After more than 6 years spent on MPC characterization and optimization of isolating procedures, we believe that these cells could facing a new era and possibly being involved in skeletal tissue engineering and regeneration. As MPCs demonstrated in vitro retaining both mesengenic and angiogenic potential, it reasonable hypothesizes the development of MPC-based implants in alternative to the modular constructs. The need of two or more different expanded cell populations giving a mesangiogenic potential to the engineered tissues could be overtaken applying cell populations like MPCs (Pacini and Petrini, 2014). However, the definition of a highly reproducible clinical-grade manufacturing process represents a first step in dealing with a possible application of MPCs in cell-based therapies. From the first report of mesangiogenic cells in human bone marrow cultures applying AS, a consistent variability in the MPC harvesting was reported. Only two out of every three samples cultured showed detectable MPC population co-isolated with MSCs, with percentages that could vary 10-fold (Petrini et al., 2009). As a consequence, we believe that the supplementation with human sera could induce considerable culture variability, during cell manufacturing process, depending on donor sex and age as well as diseases and pharmacological treatments at the time of serum collection, especially in the autologous context. Consistent production of almost pure MPC cultures has been achieved applying commercially available PhABS. Under these selective culture conditions it was possible to obtain MPCs from all the samples, with recoveries showing very low coefficient of variation (≈10%). Conversely, culturing the same samples in FBS supplemented media led to cell products constituted by MSCs only (Trombi et al., 2009). Nonetheless, we recently reported that isolation of MPCs at high grade of purity (over 95%) would be possible only after accurate screening of commercially available PhABS (Montali et al., 2016). In fact, a small number of tested batches produced cell products with consistent percentages of MSC-like cell population, compromising the purity of the MPC preparations. In order to define specific sera properties, allowing the optimal MPC manufacturing process, would be helpful the comparisons between PhABS with good performances and PhABS promoting MSC “contamination.”
Human serum contains a large and untreatable number of biological active molecules possible affecting the MPC isolation and inducing MSC proliferation (Rodrigues et al., 2010). However, recent efforts in the establishment of serum-free media for MSC in vitro expansion, demonstrated that human platelet lysate (hPL) could efficiently replace serum in culture (reviewed in Burnouf et al., 2016). This important finding narrows the choice of essential components to platelet granules content (Fekete et al., 2014). Platelets contain a lot of potent biological active molecules (Semple et al., 2011), mainly stored in the α-granules (Golebiewska and Poole, 2015), in addition to important factors involved in coagulation. These molecules include different chemokines (Semple et al., 2011) and human growth factors (hGFs), such as epidermal growth factor (EGF), basic fibroblast growth factor (FGF-2), platelet derived growth factor isoforms (PDGF-AA, -AB, and-BB), insulin-like growth factor-1 (IGF-1), vascular endothelial growth factor (VEGF), and some others (Nurden et al., 2008).
In order to define reproducible MPC manufacturing process, in the present paper we moved a first step focusing on the effect of the platelet-derived hGFs, mentioned above, on the MPC production. EGF, FGF-2, PDGFs, IGF-1, and VEGF have been reported regulating proliferation and survival of BM-derived MSCs. Thus, we hypothesize that these five hGFs or some of them could promote expansion of MSCs, during primary bone marrow cultures intended for MPC production. Here we compare six different commercial PhABS, selected on the basis of their different performance, attempting to correlate the percentage of “contaminant” MSC-like cells with the serum concentration of those hGFs.
Materials and Methods
Screening of Human Sera for MPC Isolation
The study has been performed according to the declaration of Helsinki and the sample collection protocol was approved by the ethical committee of “Azienda Ospedaliero-Universitaria Pisana”. Bone marrow aspirates were obtained after written consent from 8 patients (4M/4F, median age 65), undergoing orthopedic surgery for hip replacement. Soon after femoral neck osteotomy, approximately 10 ml of bone marrow were aspirated, using a 20 ml syringe containing 500 U.I. of heparin, and promptly sent to the cell culture facility. Samples were diluted 1:4 and hBM-MNCs collected by density gradient centrifugation using Ficoll-PaqueTMPREMIUM (GE Healthcare, Uppsala, Sweden). After two washes in Dulbecco's Modified Phosphate Buffer (D-PBS, LifeTechnologies, Carlsbad, USA-CA) cells were plated at 8 × 105/cm2 in hydrophobic six-well plates for suspension culture (Greiner Bio-One, Kremsmünster, Austria) and cultured in minimal essential medium supplemented with PhABS as previously described (Montali et al., 2016). Briefly, low-glucose Dulbecco's modified Eagle medium (DMEM, LifeTechnologies) was supplemented with 2 mM Glutamax® (LifeTechnologies), 100 μg/mL gentamicin (LifeTechnologies), and 10% PhABS. We tested six different commercially available PhABS (AB type and off-the-clot) from four different manufacturers (Table 1). Culture medium was changed every 48 h. After 7–8 days, plates were morphologically screened for MPCs using an inverted microscope, and cells subsequently detached by TrypLE® Select (LifeTechnologies) digestion and washed in D-PBS.
MSC Cell Culture
Duplicate hBM-MNC samples collected by density gradient centrifugation were plated at 2 × 105/cm2 in gas-treated culture plates for adherent cells. DMEM was supplemented with 2 mM Glutamax® (LifeTechnologies), 100 μg/mL gentamicin (LifeTechnologies), and 10% FBS (LifeTechnologies). Culture medium was changed after 48 h to remove non-adherent cells and refreshed twice a week.
Flow Cytometry
To identify MPCs and MSC-like cells (Keating, 2012) in MPC primary cultures freshly detached cells were incubated with anti-CD90 FITC-conjugated, anti-CD73 PE-conjugated, anti-CD31 PE/Cy7-conjugated, anti-CD18 APC-conjugated, and anti-CD45 VioBlue® -conjugated (Miltenyi Biotec, BergischGladbach, Germany) antibodies for 30′ at 4°C in the dark and washed twice in MACSQuant® Running Buffer (Miltenyi Biotec). Data were acquired using MACSQuant® flow cytometer and analyzed by MACSQuantify® Analysis Software (MiltenyiBiotec). MPCs were identified as CD31+CD18+CD45lowCD73negCD90neg events and MSCs as CD31negCD18negCD45negCD73brightCD90bright events. Statistical analysis was performed by one-way analysis of variance (ANOVA) test and Dunnett's post-test for multiple comparison. Results were expressed as mean value ± standard error (SE).
Gene Expression
MPCs and MSCs from primary cultures were washed twice in D-PBS, and pellets cryo-preserved in liquid nitrogen to be processed. Total RNA extraction was performed soon after thawing, using RNeasyMicro Kit (Qiage, Hilden, Germany) according to manufacturer. RNA samples (100 ng) were retro-transcribed using QuantiTect® Reverse Transcription Kit (Qiagen) and 2 μl samples of 10-fold cDNA dilutions were amplified by quantitative Real Time PCR (qRT-PCR), using iCycler-iQ5 Optical System (Bio-Rad, Hercules, USA-CA) and SsoAdvancedSYBR Green SuperMix (Bio-Rad). Samples were run in duplicate. Primer pairs (Sigma-Aldrich, St. Luis, USA-MO) were designed to detect growth factor receptor genes: BMPR1A, BMPR2, EGFR, FGFR1, FGFR2, FGFR3, IGF1R, IGF2R, KDR, PDGFRA, PDGFRB, TGFBR1, TGFBR2, and TGFBR3 (Supplementary Table S1). Relative quantitative analysis was performed following 2−ΔΔCt Livak method (Livak and Schmittgen, 2001). Normalization was performed by using RPL13A and ACTB housekeeping genes. Values were reported as log-ratios of mean MPC/MSC normalized fold expression.
Enzyme-Linked Immunosorbent Assay (ELISA)
Growth factor quantification was performed on 200 μl aliquots of serum batches, by colorimetric solid phase ELISA. In particular; epidermal growth factor (EGF), fibroblast growth factor-2 (FGF-2), and platelet-derived growth factor AB (PDGF-AB) were quantified using Quantikine® immunoassay kit (R&D Systems, Minneapolis, USA-MN). Vascular endothelial growth factor A (VEGF-A) was quantified by VEGF-A (human) BioLISA Kit Bender MedSystems® (Vienna, Austria) and insulin-like growth factor-1 (IGF-1) by IGF-1 600 ELISA kit from DRG International (Marburg, Germany). Assays were performed according to manufacturer. Percentages of MSC-like cells in MPC primary cultures were correlated with growth factor concentrations in specific sera batches by Spearman's correlation.
Recombinant hGF Treatments during MPC Isolation
hBM-MNCs were obtained from 4 patients (2M/2F, median age 68) and processed for MPC isolation as described above, applying two different sera batches: LZM, already screened for good performance and BIOW2 that produced cultures with consistent MSC-like cell “contamination.” LZM cultures were also performed in presence of 50 ng/ml rhEGF (ThermoFisher Scientific, Waltham, MA USA), 20 ng/ml rhFGF-2 (ThermoFisher Scientific), 50 ng/ml rhPDGF-AB (PeproTech EC Ltd., London, UK) or 50 ng/ml rhVEGF (ThermoFisher). Combined treatments were also performed adding rhEGF and rhFGF-2, or rhVEGF and rhPDGF-AB. In parallel BIOW2 cultures were also supplemented with 100 ng/ml rhIGF-1 (PeproTech EC Ltd., London, UK), 30 ng/ml rhIGF-2 (PeproTech EC Ltd.) alone or in combination. The culture media were changed every 2 days restoring concentrations of the hGFs. After 7 days cells were detached by TrypLE® Select digestion and processed for flow cytometry to quantify MPC and MSC percentages in the cultures, as described above. Data were collected in duplicate and reported as median values ± standard error (SE). One-way ANOVA test, coupled with Dunnett's post-test, was applied to identify significant differences.
Inhibition of hGF Receptors, during Primary Cultures
Further six bone marrow samples (4M/2F median age 68) were processed, as described above, to obtain MPC culture in BIOW2 sera batch with or without adding 4 nM of Erlotinib-HCl (OSI-744, SelleckChem, Houston, USA-TX), a potent EGFR inhibitor, and 100 nM of Nintedanib (BIBF 1120, SelleckChem) to simultaneously inhibit VEGF, FGF, and PDGF receptors. Cell cultures were performed in duplicate in six-well culture plates for suspension cultures, media were changed after 48 and 72 h restoring inhibitors concentration and at day 6 cultures were detached for flow cytometry analysis to quantify mesenchymal CD73+CD90+ population. Similarly and in parallel, MPC cultures were performed applying LZM sera batch in presence or absence of 50 nM of Linsitinib (OSI-906, SelleckChem) as IGF-1R inhibitor. Significant differences were revealed by one-way ANOVA test, and the inhibition index was calculated as difference in CD73+CD90+ percentages in treated and no-treated cultures divided by the percentage in the non-treated. Data were collected in duplicate and reported as median values ± standard error (SE).
Inhibition of hGF Receptors, during Mesengenic Differentiation of MPCs
Erlotinib-HCl and Nintedanib were also applied during mesengenic differentiation of MPCs. Briefly, MPC culture in LZM sera batch were validated for a purity higher than 95% and re-plated at 20,000 cells/cm2 in gas-treated 6-well plates for adherent cultures. After overnight incubation medium was replaced with StemMACSTM MSC expansion XF medium (Miltenyi Biotec), according to previously reported protocol (Montali et al., 2016). In parallel, mesengenic differentiation was performed in presence of 4 nM of Erlotinib-HCl and 100 nM of Nintedanib. Cultures were maintained changing medium twice a week and restoring inhibitor concentration. After 7 days mesengenic differentiation was evaluated by AlamarBlue® reduction assay (LifeTechnologies) as previously reported (Fazzi et al., 2011).
Results
We were able to isolate and grow MPCs from hBM-MNC primary cultures supplemented with all six PhABS under test. Cell yields were adequate (1.20 ± 0.23 × 105 cells/well, n = 8) to perform further analysis. Cultures supplemented with Lonza PhABS from males (LZM) or from males and females (LZMF) showed to be constituted almost exclusively by cells with the typical fried egg-shape morphology that identifies MPCs (Figure 1A; Petrini et al., 2009). Flow cytometry confirmed the characteristic CD31+CD18+CD45lowCD73negCD90neg MPC phenotype for over 95% of the cell population (red dots in Figure 1A) beside a very small CD31negCD18negCD45negCD73brightCD90bright population of MSC-like cells (blue dots in Figure 1A; Montali et al., 2016). Similar results were obtained using SeraLab PhABS (SERL) (Figure 1A). Sigma-Aldrich PhABS (SIGM) supplementation resulted in the presence of occasional spindle-shaped MSC-like cells together with a mild increase in the percentage of MSC immunophenotype (Figure 1A). Cultures supplemented with two lots of BioWest PhABS (BIOW1, BIOW2) showed a large population (10–30%) of MSC-like cells, co-isolated with MPCs. Most MSC-like cells were organized in clusters or in colonies (white arrows in Figure 1A).
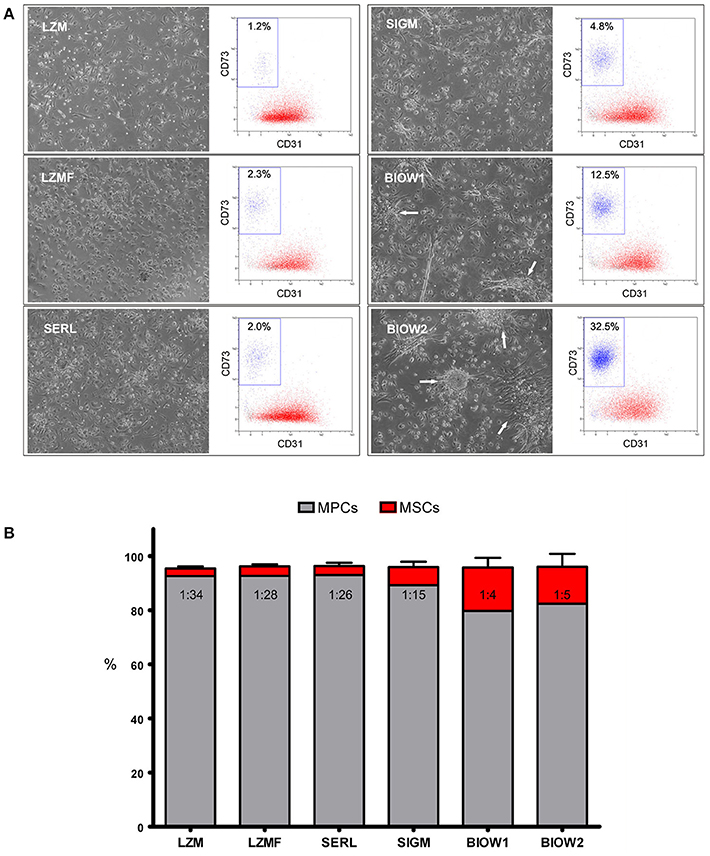
Figure 1. Analysis of different PhABS in MPC cultures from bone marrow. (A) Six different PhABS were tested for their efficiency in the isolation of MPCs. After 6 days of culture, LZM, LZMF, and SERL originated MPC cultures at high grade of purity. Cultures supplemented with SIGM showed rare MSC-like cells, while BIOW1 and BIOW2 produced a number of MSC-like cells usually clustered in proliferating foci or in rounded colonies (white arrows). Purity of cultures was estimated by flow cytometry. CD73brightCD90brightCD31neg (blue dots) percentages lower than 5.0% accounted for a good performance in MPC (red dots) production. (B) Mean MSC/MPC ratio in LZM, LZMF, and SERL varied from 1:26 to 1:34, as opposed to 1:4 and 1:5 detected in SIGM, BIOW1, and BIOW2.
We previously defined the cut point for MPC cell production as 95% of CD31+CD18+CD45lowCD73negCD90neg cells within MPC primary cultures (Montali et al., 2016). Three out of the six serum batches resulted suitable for MPC production, showing percentages of MSC-like cells lower than 5% with no significant variation: LZM (2.7 ± 0.6%), LZMF (3.3 ± 0.5%), SERL (3.3 ± 1.2%). SIGM was borderline (5.9 ± 1.4%), while BIOW1 and BIOW2 turned out not to be fitting because of significantly (p < 0.01 to LZM, p < 0.05 to LZMF and SERL) higher percentages of MSC-like cells (17.7 ± 4.8% and 16.2 ± 3.9%, respectively) resulting in MSC/MPC frequencies around 1:4–1:5 (Figure 1B).
MPCs from LZM supplemented cultures were selectively chosen for gene expression analysis of growth factor receptors in comparison to standard FBS-cultured MSCs. Expression of 3 out of the 14 genes analyzed was over one log higher in MSCs: FGFR2 (−1.74 log, p < 0.05, n = 8), PDGFRA (−1.58 log, p < 0.05, n = 8), and EGFR (−1.33 log, p < 0.01, n = 8). PDGFRB and TGFR3 were also significantly more expressed in MSCs although at lower levels (−0.90 and −0.69 log, respectively, p < 0.05, n = 8). Conversely, IGF2R and KDR expression was significantly higher in MPCs, with IGF2R only over one log higher (1.27 log, p < 0.01, n = 8, Figure 2). Higher levels of IGF1R and TGFRB2 were detected in MPCs with a confidence limit lower than 90% (0.37 log, p = 0.373 and 0.51 log, p = 0.198, n = 8, respectively). BMPR1A, BMPR2, FGFR1, FGFR3, and TGFRB1 showed no significant differences.
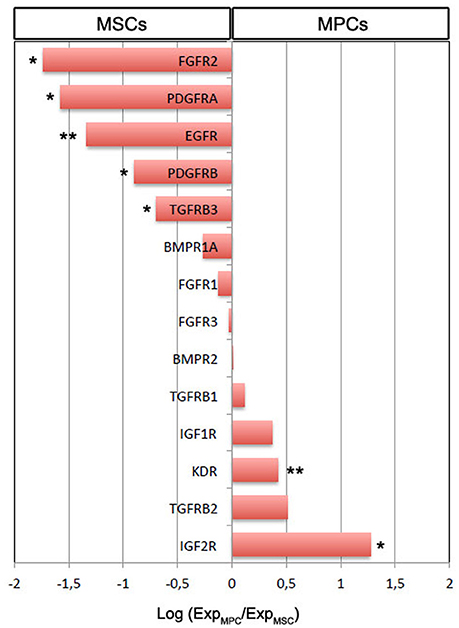
Figure 2. Gene expression analysis of growth factor receptor genes in MSCs vs. MPCs. The majority of the receptor genes resulted differentially expressed (*p < 0.05, **p < 0.01). Expression of FGFR2, PDGFRA, and EGFR was over one log higher in MSCs. Conversely, 10-fold higher IGF2R expression was evidenced in MPCs.
The “contamination” of MSC-like cells in MPC primary cultures was correlated to the concentration of growth factors in the different sera. Higher concentrations of EGF, FGF-2, PDGF-AB and VEGF-A were consistently found in BIOW1 and BIOW2 that produced cultures with higher percentages of MSC-like cells (Table 2). FGF-2 revealed a dose-dependent correlation showing a Spearman ratio of 0.943 (p < 0.05, n = 8, Figure 3). In order to confirm the involvement of these hGFs in the poor performance of a pooled sera batch, we separately added consistent amount these factors to LZM supplemented cultures. Percentages of MSC-like cells resulted significantly increased in the rhEGF (34.3 ± 14.0%, p < 0.01, n = 4) and rhFGF-2 (39.2 ± 17.5%, p < 0.01, n = 4) treated primary cultures, respect to LZM control (4.6 ± 1.7%, n = 4, Figure 4). Combining these two hGFs produced similar results (40.5 ± 17.9%, n = 4), with no significant differences respect to cultures treated with EGF or FGF-2 separately. Conversely, adding rhVEGF (5.6 ± 1.4%, n = 4) or rhPDGF-AB (6.4 ± 0.5%, n = 4) resulted having no significant effects on MPC cultures when added separately. Combination of those hGF instead showed a mild increase in MSC-like cell percentages (11.3 ± 0.3%, p < 0.01, n = 4), resulting in cultures with purity lower than the cut-off point established for feasible MPC products.
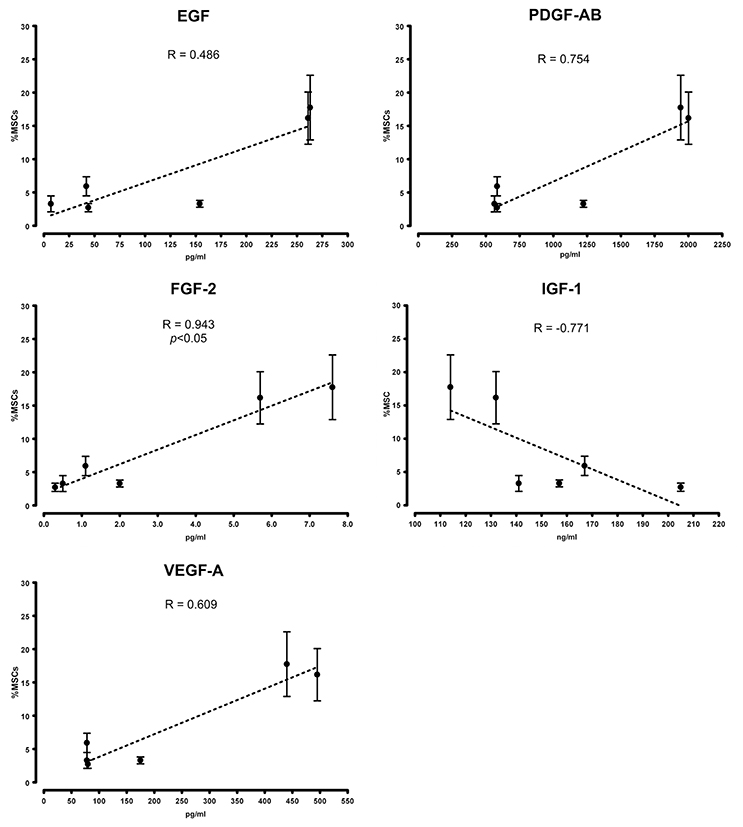
Figure 3. Growth factor serum content correlated with cell composition in primary cultures. Higher concentrations of EGF, FGF-2, PDGF-AB, and VEGF-A were detected in PhABS that gave origin to cultures with higher percentages of “contaminating” MSC-like cells. Good performance in the initiation of MPC cultures at high grade of purity correlated with elevated levels of IGF-1.
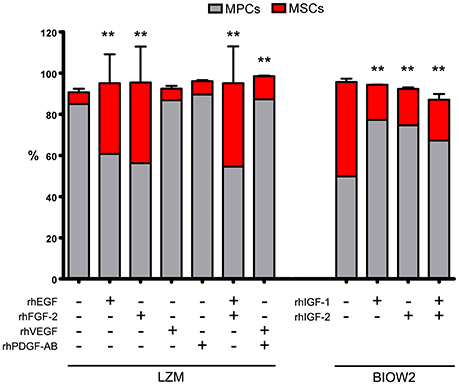
Figure 4. Managing hGF medium contents, by adding recombinant factors, affected the sera performance during MPC isolation. Adding rhEGF or rhFGF-2, alone or in combination, to the cultures supplemented with good performance serum (LZM), resulted in significantly increased MSC-like population (red bars). Under these conditions, MPC/MSC ratio was comparable to those reported applying MSC-inducing PhABS. Treating with rhVEGF or rhPDGF-AB had no effects on MPC isolation, but a combination of those two hGFs produced mild but significant increase in MSC-like counterpart. BIOW2 batch was selected as PhABS with poor performance, evaluating the effects of rhIGF-1 and rhIGF-2 during MPC isolation. The treatment with rhIGFs, either alone or in combination, produced a 50% reduction of the MSC-like population (**p < 0.01, respect to control).
Medium supplemented with BIOW2 was selected in order to verify if higher concentration of IGFs could be related to the good performances of feasible sera batches. A consistent reduction, around 50%, of the MSC “contamination” was reported in rhIGF-1 (17.1 ± 0.1%, p < 0.01, n = 4) and rhIGF-2 (17.7 ± 0.2%, p < 0.01, n = 4) treated cultures, alone or in combination (19.8 ± 2.8%, p < 0.01, n = 4) respect to BIOW2 control (45.8 ± 1.6%, n = 4, Figure 4).
The effect of high concentration of EGF, FGF-2, VEGF and PDGFs in the poor performance of pooled sera batches, during MPC production, was also confirmed by receptor inhibition experiments. In fact, the addition of Erlotinib-HCl and Nintedanib to BIOW2 cultures resulted in the 50% reduction of the CD73+CD90+ population (mean inhibition index: 54.2 ± 7.7%, p < 0.05, n = 6) at the primary cultures, producing suitable MPC products (>95% of purity) comparable to ones obtained applying LZM (Figure 5A). Elevated levels of IGF-1 were instead detected in the sera batches with low mesenchymal contamination (LZM, LZMF) but the inhibition of its receptors did not produce any detectable effect during primary culture (Figure 5B).
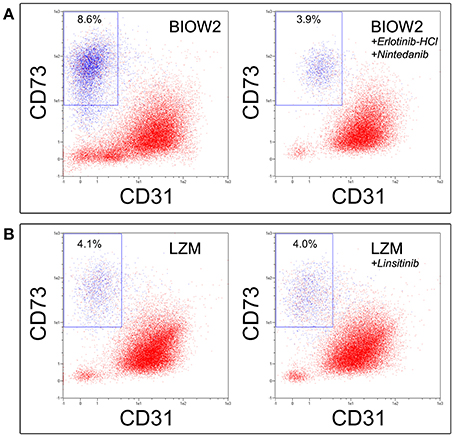
Figure 5. Erlotinib-HCl in combination with Nintedanib allowed recovering quality MPC products applying sera with poor performance. (A) The simultaneous inhibition of EGFR, FGFRs, PDGFRs and VEGFRs during culture of hBM-MNCs in BIOW2 serum batch, produce a 50% reduction in the CD73+CD90+ population, leading to a cell product comparable to once produced in LZM. (B) Conversely, the inhibition of IGF-1R exerted by Linsitinib did not affect the MPC production.
Erlotinib-HCl and Nintenadib also affected the in vitro MPC mesengenic differentiation, with a decreased AlamarBlue® reduction from 20 to 40% respect to control (mean inhibition index: 22.7 ± 10.9%, p < 0.05, n = 6). Conversely, Linsitinib produced no detectable effects (data not shown).
Discussion
Ex vivo expansion of bone marrow-derived multipotent cells requires supplementation of basal culture medium in order to sustain cell proliferation while maintaining cell differentiation capability (Bernardo et al., 2011). FBS-based expansion protocols have been used in the very first clinical applications of these promising cells (Le Blanc et al., 2004, 2008). However, safety concerns have been raised regarding use of supplements of animal origin in clinical-grade expansion protocols (Sensebé and Bourin, 2008; Reinhardt et al., 2011; Sensebé et al., 2011). Consequently, many efforts have been made to replace animal derived supplements and reagents with human blood derivatives as autologous serum (AS) (Stute et al., 2004), pooled human AB serum (PhABS) (Bieback et al., 2009), platelet lysate (PL) (Hemeda et al., 2014) or a combination of them (Kocaoemer et al., 2007; Muraglia et al., 2015).
In this view, during the last years our group has been focusing on the definition of a clinical grade protocol to culture bone marrow-derived multipotent cells by replacing FBS with AS or PhABS. Our efforts led to the first description of mesangiogenic progenitor cells (MPCs) as previously not described cell population, co-isolated in AS-supplemented MSC cultures (Petrini et al., 2009). In parallel, a group of colleagues demonstrated the long-term efficacy and safety of this kind of cell products for the healing of atrophic pseudarthrosis of the upper limb (Giannotti et al., 2013). In this paper, Authors also hypothesized that MPCs detected in the applied cell populations, even if at low percentage (1–10%), could contribute to the long lasting healing (follow-up at 76 months) reported for the eight enrolled patients. This interesting hypothesis could be corroborated by the idea that implanted MPCs could provide efficient tissue regeneration, differentiating into early MSCs, as well as contribute to the vascularization of the engineered construct due to their demonstrated angiogenic properties (Fazzi et al., 2011). After these encouraging clinical results, we believe that manufacturing MPC-based medicinal products could provide a new promising tool in skeletal tissue engineering.
As MPCs have been reported being not-proliferating cells, the expansion is not actually permitted. Nonetheless, the frequency of MPCs has been estimated around 1% of hBM-MNCs, which is hundred to thousand times higher respect to MSCs (Trombi et al., 2009), leading us to hypothesized cell-based therapies involving this non-expanded multipotent progenitors. The possible application of not in vitro amplified cell products carries significant advantages in terms of: (i) a reduced risk of cell transformation, (ii) reduced cellular senescence, and (iii) reduced exposition to bacterial and viral contamination, minimizing the culture time (Schneider et al., 2010). However, this could provide limited number of cell doses forcing to investigate any possible clinical application of MPCs in the context of personalized cell therapies, which involved small-scale CBMP production similar to that described by Giannotti et al. (2013). Anyway, in the attempt to define manufacturing conditions, selective for MPCs, we observed large variability in MPC yield using AS as well as different manufacturers and batches of commercial PhABS.
In general, CBMPs represent complex biological products displaying high rate of intrinsic variability, mainly derived from two sources: (i) process starting material and (ii) process conditions (Williams et al., 2012). In particular for MPC-based product, the first mentioned source of variability could not be eliminated in the autologous context where starting materials are obtained by different patients. Moreover, even if the variability related to the production process could be significantly reduced applying automated and well-controlled manufacturing process (Liu et al., 2010; Pacini, 2014), the serum supplementation represents an inescapable source of variability. Here we showed such variability to correlate with some of the most important growth factors contained in platelet lysates (Fekete et al., 2012), whose concentrations in the serum fluctuate regardless of donors' age, sex or production method. Best performance in MPC production was obtained with serum concentrations of IGF-1 over 150 ng/ml combined to low levels of EGF (<160 pg/ml), FGF-2 (<2.0 pg/ml), PDGF-AB (<1200 pg/ml), and VEGF-A (<180 pg/ml). Screening these five growth factors could provide a simple means to select high quality HS batches for the production of MPC-based CBMPs. Moreover, data presented here also provide interesting application for Erlotinib-HCl and Nintedanib in the production of MPC in autologous serum, where any possible source of variability, related to fluctuations in hGF serum concentration, could be mitigated by receptors inhibition. This could possibly results in qualified MPC products independently by patient sex, age, etc.
Taking in consideration only hGFs as serum factors influencing MPC cultures, represents the mayor limit of this study. Serum is highly complex biological fluid composed also by many other macromolecules (i.e., cytokines and immunoglobulins), different lipids, hormones and other small molecules. Further investigations are needed to exhaustively define the essential components required to produce high quality MPC-based cell product. Anyway, here we clearly demonstrate that managing the interactions of these hGFs with their receptors could also represents a new tool in MSC production. In fact, culturing bone marrow in AS, or PhABS, and in presence of Erlotinib-HCl and Nintedanib could lead to highly purified MPCs that could act as culture initiating cells to produce more homogeneous and synchronized MSC cultures. Those cultures could be easily obtained applying the same HS, by removing receptor inhibition and supplementing with recombinant hGF cocktail including at least EGF and FGF-2, as demonstrated here. Our findings are in accordance with Yamaguchi et al. that reported comparable results between FBS and PhABS, in MSC culture, when PhABS cultures had been supplemented with 10 ng/ml of FGF-2 (Yamaguchi et al., 2002). However, the extensive literature on serum supplements in MSC cultures is very controversial. A number of authors showed no significant differences between AS and FBS (Yamamoto et al., 2003; Spees et al., 2004; Stute et al., 2004), while Shigeno and Ashton obtained greater response using AS (Shigeno and Ashton, 1995), in contrast to the extensively reported higher performances applying FBS (Koller et al., 1998; Kuznetsov et al., 2000). It is reasonable hypothesized that the origins of those controversial data, in MSC manufacturing, reside in the lack of preliminary characterization of hGF content in the HS applied. We also believe that those inconsistencies originate from restricting focus on MSCs, without taking into account the positive effects of the MPC presence within the CBMP once implanted in vivo.
HS batches rich in EGF and FGF-2 apparently worsen the production of standard monomorphic MPC cultures probably supporting the proliferation of MSC-like cells from other distinct and rare progenitors reported in the bone marrow. These mesengenic progenitors could include skeletal stem cells (SSCs), recently described as the genuine skeletal tissue stem cells (Bianco and Robey, 2015) or CD146-positive non-stem osteoprogenitor (Sacchetti et al., 2007). This hypothesis is validated by our data on growth factor receptor expression. Significantly higher levels of EGFR, FGFR2, and PDGFRA were reported in MSCs suggesting that these specific growth factors could have trophic effect on MSC-like cells (Ng et al., 2008) instead of inducing MPCs to differentiate. Conversely, VEGF-A was detected at higher concentrations in MSC-inducing sera while KDR was more expressed in MPCs, together with TGFBR2, suggesting a role for VEGF-A and possibly TGF-β in MPC differentiation toward the mesenchymal lineage. IGF-1 appeared to be acting as MPC-promoting factor as its levels were particularly high in MPC-inducing sera LZM, LZMF, and SERL, mirroring IGF receptor expression in MPCs.
In conclusion, we believe that MPCs represent also a promising alternative to hBM-MNCs as culture-initiating cells in the production of clinical grade MSCs. A purified and well-characterized progenitor cell population cultured under specific controlled conditions would significantly improve CBMP reproducibility and consequently the predictability of pre-clinical studies. MPCs are found at frequencies one to two logs higher than the other MSC progenitors described in bone marrow. Moreover, future clinical applications for CBMPs based on undifferentiated and not expanded MPCs could take advantage from their angiogenic potential that is suggestive of possible beneficial effects on neo- or re-vascularization of target tissues. A complete definition of the active growth factor cocktail for MPC efficient isolation, expansion, and differentiation is still required also in order to possibly develop specific chemically defined media (CDM), which will eliminate the biological variability related to the serum supplementation.
Author Contributions
MM: Conception and design, Data collection, assembly, analysis, and interpretation. SB: Data collection, assembly, analysis, and interpretation. VC: Collection and assembly of RT-PCR data. FF: Collection and assembly of ELISA assay data. FP: Data collection. IP: Conception and design. SP: Conception and design; Data collection, assembly, analysis, and interpretation; Manuscript writing.
Funding
This work was funded by “Centro per l'Uso Clinico delle Cellule Staminali” (CUCCS) as part of the project “Impiego di cellule stromali mesenchimali di origine midollare nelle pseudoatrosi, cisti ossee di astragalo e osteotomie in plus delle ossa lunghe” (project number 539999_2014_Petrini_CUCCS).
Conflict of Interest Statement
The authors declare that the research was conducted in the absence of any commercial or financial relationships that could be construed as a potential conflict of interest.
Acknowledgments
Authors would like to thank Dr. Paolo Parchi from the 1st Orthopedic Division of the department of Translational Research and New Technology in Medicine and Surgery, University of Pisa, for providing high quality bone marrow samples.
Supplementary Material
The Supplementary Material for this article can be found online at: https://www.frontiersin.org/article/10.3389/fcell.2016.00114
References
Altaie, A., Owston, H., and Jones, E. (2016). Use of platelet lysate for bone regeneration - are we ready for clinical translation? World J. Stem Cells 8, 47–55. doi: 10.4252/wjsc.v8.i2.47
Bernardo, M. E., Cometa, A. M., Pagliara, D., Vinti, L., Rossi, F., Cristantielli, R., et al. (2011). Ex vivo expansion of mesenchymal stromal cells. Best Pract. Res. Clin. Haematol. 24, 73–81. doi: 10.1016/j.beha.2010.11.002
Bianco, P., and Robey, P. G. (2015). Skeletal stem cells. Development 142, 1023–1027. doi: 10.1242/dev.102210
Bieback, K., Hecker, A., Kocaömer, A., Lannert, H., Schallmoser, K., Strunk, D., et al. (2009). Human alternatives to fetal bovine serum for the expansion of mesenchymal stromal cells from bone marrow. Stem Cells 27, 2331–2341. doi: 10.1002/stem.139
Burnouf, T., Strunk, D., Koh, M. B., and Schallmoser, K. (2016). Human platelet lysate: replacing fetal bovine serum as a gold standard for human cell propagation? Biomaterials 76, 371–387. doi: 10.1016/j.biomaterials.2015.10.065
Butler, M. J., and Sefton, M. V. (2012). Cotransplantation of adipose-derived mesenchymal stromal cells and endothelial cells in a modular construct drives vascularization in SCID/bg mice. Tissue Eng. Part A. 18, 1628–1641. doi: 10.1089/ten.tea.2011.0467
Chamberlain, M. D., West, M. E., Lam, G. C., and Sefton, M. V. (2015). In vivo remodelling of vascularizing engineered tissues. Ann. Biomed. Eng. 43, 1189–1200. doi: 10.1007/s10439-014-1146-x
Crisan, M., Yap, S., Casteilla, L., Chen, C.-W., Corselli, M., Park, T. S., et al. (2008). A perivascular origin for mesenchymal stem cells in multiple human organs. Cell Stem Cell 3, 301–313. doi: 10.1016/j.stem.2008.07.003
da Silva Meirelles, L., Chagastelles, P. C., and Nardi, N. B. (2006). Mesenchymal stem cells reside in virtually all post-natal organs and tissues. J. Cell Sci. 119, 2204–2213. doi: 10.1242/jcs.02932
Fazzi, R., Pacini, S., Carnicelli, V., Trombi, L., Montali, M., Lazzarini, E., et al. (2011). Mesodermal progenitor cells (MPCs) differentiate into mesenchymal stromal cells (MSCs) by activation of Wnt5/calmodulin signalling pathway. PLoS ONE 6:e25600. doi: 10.1371/journal.pone.0025600
Fekete, N., Gadelorge, M., Fürst, D., Maurer, C., Dausend, J., Fleury-Cappellesso, S., et al. (2012). Platelet lysate from whole blood-derived pooled platelet concentrates and apheresis-derived platelet concentrates for the isolation and expansion of human bone marrow mesenchymal stromal cells: production process, content and identification of active components. Cytotherapy 14, 540–554. doi: 10.3109/14653249.2012.655420
Fekete, N., Rojewski, M. T., Lotfi, R., and Schrezenmeier, H. (2014). Essential components for ex vivo proliferation of mesenchymal stromal cells. Tissue Eng. C Methods 20, 129–139. doi: 10.1089/ten.tec.2013.0061
Giannotti, S., Trombi, L., Bottai, V., Ghilardi, M., D'Alessandro, D., Danti, S., et al. (2013). Use of autologous human mesenchymal stromal cell/fibrin clot constructs in upper limb non-unions: long-term assessment. PLoS ONE 8:e73893. doi: 10.1371/journal.pone.0073893
Golebiewska, E. M., and Poole, A. W. (2015). Platelet secretion: from haemostasis to wound healing and beyond. Blood Rev. 29, 153–162. doi: 10.1016/j.blre.2014.10.003
Hak, D. J., Fitzpatrick, D., Bishop, J. A., Marsh, J. L., Tilp, S., Schnettler, R., et al. (2014). Delayed union and nonunions: epidemiology, clinical issues, and financial aspects. Injury 45, S3–S7. doi: 10.1016/j.injury.2014.04.002
Haynesworth, S. E., Goshima, J., Goldberg, V. M., and Caplan, A. I. (1992). Characterization of cells with osteogenic potential from human marrow. Bone 13, 81–88. doi: 10.1016/8756-3282(92)90364-3
Hemeda, H., Giebel, B., and Wagner, W. (2014). Evaluation of human platelet lysate versus fetal bovine serum for culture of mesenchymal stromal cells. Cytotherapy 16, 170–180. doi: 10.1016/j.jcyt.2013.11.004
Herberts, C. A., Kwa, M. S., and Hermsen, H. P. (2011). Risk factors in the development of stem cell therapy. J. Transl. Med. 9:29. doi: 10.1186/1479-5876-9-29
Hutton, D. L., and Grayson, W. L. (2014). Stem cell-based approaches to engineering vascularized bone. Curr. Opin. Chem. Eng. 3, 75–82. doi: 10.1016/j.coche.2013.12.002
Keating, A. (2012). Mesenchymal stromal cells: new directions. Cell Stem Cell 10, 709–716. doi: 10.1016/j.stem.2012.05.015
Kirton, J. P., and Xu, Q. (2010). Endothelial precursors in vascular repair. Microvasc. Res. 79, 193–199. doi: 10.1016/j.mvr.2010.02.009
Kocaoemer, A., Kern, S., Klüter, H., and Bieback, K. (2007). Human AB serum and thrombin-activated platelet-rich plasma are suitable alternatives to fetal calf serum for the expansion of mesenchymal stem cells from adipose tissue. Stem Cells 25, 1270–1278. doi: 10.1634/stemcells.2006-0627
Koller, M. R., Maher, R. J., Manchel, I., Oxender, M., and Smith, A. K. (1998). Alternatives to animal sera for human bone marrow cell expansion: human serum and serum-free media. J. Hematother. 7, 413–423. doi: 10.1089/scd.1.1998.7.413
Kuznetsov, S. A., Mankani, M. H., and Robey, P. G. (2000). Effect of serum on human bone marrow stromal cells: ex vivo expansion and in vivo bone formation. Transplantation 70, 1780–1787. doi: 10.1097/00007890-200012270-00018
Le Blanc, K., Frassoni, F., Ball, L., Locatelli, F., Roelofs, H., Lewis, I., et al. (2008). Mesenchymal stem cells for treatment of steroid-resistant, severe, acute graft-versus-host disease: a phase II study. Lancet 371, 1579–1586. doi: 10.1016/S0140-6736(08)60690-X
Le Blanc, K., Rasmusson, I., Sundberg, B., Götherström, C., Hassan, M., Uzunel, M., et al. (2004). Treatment of severe acute graft-versus-host disease with third party haploidentical mesenchymal stem cells. Lancet 363, 1439–1441. doi: 10.1016/S0140-6736(04)16104-7
Lechanteur, C., Briquet, A., Giet, O., Delloye, O., Baudoux, E., and Beguin, Y. (2016). Clinical-scale expansion of mesenchymal stromal cells: a large banking experience. J. Transl. Med. 20, 145. doi: 10.1186/s12967-016-0892-y
Liu, Y., Hourd, P., Chandra, A., and Williams, D. J. (2010). Human cell culture process capability: a comparison of manual and automated production. J. Tissue Eng. Regen. Med. 4, 45–54. doi: 10.1002/term.217
Livak, K. J., and Schmittgen, T. D. (2001). Analysis of relative gene expression data using real-time quantitative PCR and the 2−ΔΔCT method. Methods 25, 402–408. doi: 10.1006/meth.2001.1262
Montali, M., Barachini, S., Pacini, S., Panvini, F. M., and Petrini, M. (2016). Isolating mesangiogenic progenitor cells (MPCs) from human bone marrow. J. Vis. Exp. 15, 113. doi: 10.3791/54225
Muraglia, A., Todeschi, M. R., Papait, A., Poggi, A., Spanò, R., Strada, P., et al. (2015). Combined platelet and plasma derivatives enhance proliferation of stem/progenitor cells maintaining their differentiation potential. Cytotherapy 17, 1793–1806. doi: 10.1016/j.jcyt.2015.09.004
Murray, I. R., West, C. C., Hardy, W. R., James, A. W., Park, T. S., Nguyen, A., et al. (2014). Natural history of mesenchymal stem cells, from vessel walls to culture vessels. Cell. Mol. Life Sci. 71, 1353–1374. doi: 10.1007/s00018-013-1462-6
Ng, F., Boucher, S., Koh, S., Sastry, K. S., Chase, L., Lakshmipathy, U., et al. (2008). PDGF, TGF-β, and FGF signaling is important for differentiation and growth of mesenchymal stem cells (MSCs): transcriptional profiling can identify markers and signaling pathways important in differentiation of MSCs into adipogenic, chondrogenic, and osteogenic lineages. Blood 112, 295–307. doi: 10.1182/blood-2007-07-103697
Nurden, A. T., Nurden, P., Sanchez, M., Andia, I., and Anitua, E. (2008). Platelets and wound healing. Front. Biosci. 1, 3532–3548. doi: 10.2741/2947
Pacini, S. (2014). Deterministic and stochastic approaches in the clinical application of mesenchymal stromal cells (MSCs). Front. Cell Dev. Biol. 2:50. doi: 10.3389/fcell.2014.00050
Pacini, S., Carnicelli, V., Trombi, L., Montali, M., Fazzi, R., Lazzarini, E., et al. (2010). Constitutive expression of pluripotency-associated genes in mesodermal progenitor cells (MPCs). PLoS ONE 5:e9861. doi: 10.1371/journal.pone.0009861
Pacini, S., and Petrini, I. (2014). Are MSCs angiogenic cells? New insights on human nestin-positive bone marrow-derived multipotent cells. Front. Cell Dev. Biol. 2:20. doi: 10.3389/fcell.2014.00020
Pacini, S., Spinabella, S., Trombi, L., Fazzi, R., Galimberti, S., Dini, F., et al. (2007). Suspension of bone marrow–derived undifferentiated mesenchymal stromal cells for repair of superficial digital flexor tendon in race horses. Tissue Eng. 13, 2949–2955. doi: 10.1089/ten.2007.0108
Pelosi, E., Castelli, G., and Testa, U. (2014). Endothelial progenitors. Blood Cells Mol. Dis. 52, 186–194. doi: 10.1016/j.bcmd.2013.11.004
Petrini, M., Pacini, S., Trombi, L., Fazzi, R., Montali, M., Ikehara, S., et al. (2009). Identification and purification of mesodermal progenitor cells from human adult bone marrow. Stem Cells Dev. 18, 857–866. doi: 10.1089/scd.2008.0291
Prockop, D. J. (1997). Marrow stromal cells as stem cells for nonhematopoietic tissues. Science 276, 71–74. doi: 10.1126/science.276.5309.71
Rao, R. R., and Stegemann, J. P. (2013). Cell-based approaches to the engineering of vascularized bone tissue. Cytotherapy 15, 1309–1322. doi: 10.1016/j.jcyt.2013.06.005
Reinhardt, J., Stühler, A., and Blümel, J. (2011). Safety of bovine sera for production of mesenchymal stem cells for therapeutic use. Hum. Gene Ther. 22, 775; author reply 776. doi: 10.1089/hum.2011.2501
Richardson, S. M., Kalamegam, G., Pushparaj, P. N., Matta, C., Memic, A., Khademhosseini, A., et al. (2015). Mesenchymal stem cells in regenerative medicine: focus on articular cartilage and intervertebral disc regeneration. Methods 99, 69–80. doi: 10.1016/j.ymeth.2015.09.015
Rodrigues, M., Griffith, L. G., and Wells, A. (2010). Growth factor regulation of proliferation and survival of multipotential stromal cells. Stem Cell Res. Ther. 26:32. doi: 10.1186/scrt32
Sacchetti, B., Funari, A., Michienzi, S., Di Cesare, S., Piersanti, S., Saggio, I., et al. (2007). Self-renewing osteoprogenitors in bone marrow sinusoids can organize a hematopoietic microenvironment. Cell 131, 324–336. doi: 10.1016/j.cell.2007.08.025
Schneider, C. K., Salmikangas, P., Jilma, B., Flamion, B., Todorova, L. R., Paphitou, A., et al. (2010). Challenges with advanced therapy medicinal products and how to meet them. Nat. Rev. Drug Discov. 9, 195–201. doi: 10.1038/nrd3052
Semple, J. W., Italiano, J. E. Jr., and Freedman, J. (2011). Platelets and the immune continuum. Nat. Rev. Immunol. 11, 264–274. doi: 10.1038/nri2956
Sensebé, L., and Bourin, P. (2008). Producing MSC according GMP: process and controls. Biomed. Mater. Eng. 18, 173–177. doi: 10.3233/BME-2008-0520
Sensebé, L., Bourin, P., and Tarte, K. (2011). Good manufacturing practices production of mesenchymal stem/stromal cells. Hum. Gene Ther. 22, 19–26. doi: 10.1089/hum.2010.197
Shigeno, Y., and Ashton, B. (1995). Human bone-cell proliferation in vitro decreases with human donor age. J. Bone Joint. Surg. Br. 77, 139–142.
Spees, J. L., Gregory, C. A., Singh, H., Tucker, H. A., Peister, A., Lynch, P. J., et al. (2004). Internalized antigens must be removed to prepare hypoimmunogenic mesenchymal stem cells for cell and gene therapy. Mol. Ther. 9, 747–756. doi: 10.1016/j.ymthe.2004.02.012
Stute, N., Holtz, K., Bubenheim, M., Lange, C., Blake, F., and Zander, A. R. (2004). Autologous serum for isolation and expansion of human mesenchymal stem cells for clinical use. Exp. Hematol. 32, 1212–1225. doi: 10.1016/j.exphem.2004.09.003
Subbanna, P. K. (2007). Mesenchymal stem cells for treating GVHD: in vivo fate and optimal dose. Med. Hypotheses 69, 469–470. doi: 10.1016/j.mehy.2006.12.016
Trombi, L., Pacini, S., Montali, M., Fazzi, R., Chiellini, F., Ikehara, S., et al. (2009). Selective culture of mesodermal progenitor cells. Stem Cells Dev. 18, 1227–1234. doi: 10.1089/scd.2009.0054
Watson, L., Elliman, S. J., and Coleman, C. M. (2014). From isolation to implantation: a concise review of mesenchymal stem cell therapy in bone fracture repair. Stem Cell Res. Ther. 5:51. doi: 10.1186/scrt439
Williams, D. J., Thomas, R. J., Hourd, P. C., Chandra, A., Ratcliffe, E., Liu, Y., et al. (2012). Precision manufacturing for clinical-quality regenerative medicines. Philos. Trans. A Math. Phys. Eng. Sci. 370, 3924–3949. doi: 10.1098/rsta.2011.0049
Yamaguchi, M., Hirayama, F., Wakamoto, S., Fujihara, M., Murahashi, H., Sato, N., et al. (2002). Bone marrow stromal cells prepared using AB serum and bFGF for hematopoietic stem cells expansion. Transfusion 42, 921–927. doi: 10.1046/j.1537-2995.2002.00149.x
Yamamoto, N., Isobe, M., Negishi, A., Yoshimasu, H., Shimokawa, H., Ohya, K., et al. (2003). Effects of autologous serum on osteoblastic differentiation in human bone marrow cells. J. Med. Dent. Sci. 50, 63–69.
Yousefi, A. M., James, P. F., Akbarzadeh, R., Subramanian, A., Flavin, C., and Oudadesse, H. (2016). Prospect of stem cells in bone tissue engineering: a review. Stem Cells Int. 2016:6180487. doi: 10.1155/2016/6180487
Keywords: mesangiogenic progenitor cells, mesenchymal stromal cells, human serum, growth factors, bone marrow culture, cell-based medicinal product, Erlotinib, Nintedanib
Citation: Montali M, Barachini S, Panvini FM, Carnicelli V, Fulceri F, Petrini I and Pacini S (2016) Growth Factor Content in Human Sera Affects the Isolation of Mesangiogenic Progenitor Cells (MPCs) from Human Bone Marrow. Front. Cell Dev. Biol. 4:114. doi: 10.3389/fcell.2016.00114
Received: 12 July 2016; Accepted: 29 September 2016;
Published: 17 October 2016.
Edited by:
Erdal Karaoz, Liv Hospital, TurkeyReviewed by:
Aaron W. James, University of California, Los Angeles, USANedime Serakinci, Near East University, Turkey
Copyright © 2016 Montali, Barachini, Panvini, Carnicelli, Fulceri, Petrini and Pacini. This is an open-access article distributed under the terms of the Creative Commons Attribution License (CC BY). The use, distribution or reproduction in other forums is permitted, provided the original author(s) or licensor are credited and that the original publication in this journal is cited, in accordance with accepted academic practice. No use, distribution or reproduction is permitted which does not comply with these terms.
*Correspondence: Simone Pacini, simone.pacini@do.unipi.it